Science laboratory technology is revolutionizing scientific research and discovery. From the evolution of microscopes to the integration of artificial intelligence, advancements in lab technology are accelerating the pace of innovation across diverse scientific fields. This exploration delves into the multifaceted world of modern laboratories, examining the equipment, techniques, and safety protocols that drive progress in scientific understanding.
This examination covers the evolution of laboratory equipment, the role of automation and data analysis, safety and security measures, the impact of robotics, and the implementation of Laboratory Information Management Systems (LIMS). Furthermore, we’ll explore the growing significance of virtual laboratories, sustainable practices, and the transformative potential of emerging diagnostic technologies. The future of science laboratory technology holds immense promise, shaping the trajectory of scientific exploration and technological advancement.
Laboratory Equipment Advancements
The evolution of laboratory equipment has been a driving force behind scientific progress, enabling increasingly sophisticated research and analysis. This advancement is particularly evident in microscopy and the growing role of automation, paving the way for even more innovative technologies in the future.
Microscope Evolution
Microscopes have undergone a dramatic transformation over the last century. From relatively simple optical instruments, they’ve evolved into highly complex and specialized tools capable of visualizing structures at the nanoscale. The development of electron microscopy, for instance, revolutionized biological and materials science, allowing researchers to visualize structures far smaller than those visible with light microscopes. This progress has been driven by continuous improvements in lens design, illumination techniques, and image processing capabilities.
Microscope Type | Magnification | Resolution | Applications |
---|---|---|---|
Optical (Brightfield) | Up to 1500x | ~200 nm | General cell observation, histology |
Phase-Contrast | Up to 1000x | ~200 nm | Observing transparent specimens, live cells |
Fluorescence | Up to 1500x | ~200 nm | Immunofluorescence, localization of specific molecules |
Transmission Electron (TEM) | Up to 1,000,000x | ~0.1 nm | High-resolution imaging of ultrastructures, materials science |
Automation in Laboratory Procedures
Automation has significantly impacted laboratory procedures, increasing throughput, improving accuracy, and reducing human error. Liquid handling robots, automated analyzers, and high-throughput screening systems are now commonplace in many laboratories. For example, automated DNA sequencing machines have drastically sped up genomic research, enabling large-scale projects that were previously inconceivable. The integration of robotics and software allows for complex experiments to be performed with greater precision and consistency, leading to more reliable results and faster turnaround times.
Hypothetical Future Laboratory
A future laboratory could integrate nanotechnology and AI to create a highly efficient and intelligent research environment. Nanomaterials could be used to create highly sensitive sensors and actuators, enabling real-time monitoring and control of experiments. AI algorithms could analyze vast datasets generated by experiments, identify patterns and anomalies, and even design new experiments based on previous results. Imagine a laboratory where nanoscale robots perform intricate procedures autonomously, guided by AI, while sophisticated sensors provide constant feedback, minimizing the risk of error and maximizing efficiency. Such a laboratory would accelerate scientific discovery across diverse fields, pushing the boundaries of our understanding of the world around us. This concept aligns with the development of microfluidic devices and lab-on-a-chip technologies, which already miniaturize and automate many laboratory processes.
Data Acquisition and Analysis in Science Labs
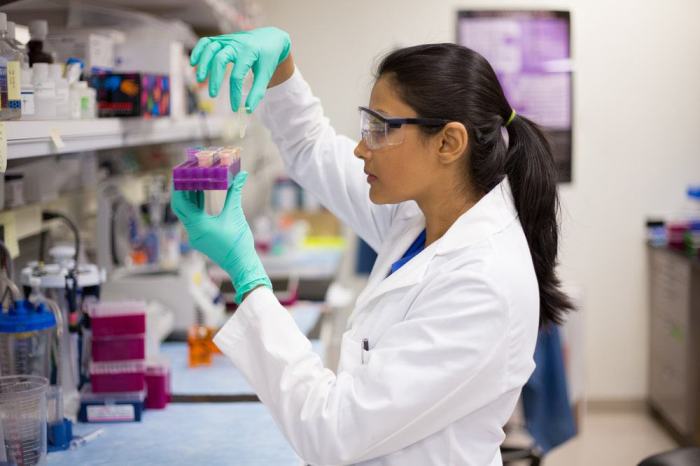
The efficient collection and analysis of data are fundamental to the success of any scientific investigation. Modern science relies heavily on sophisticated techniques and technologies to ensure accuracy, reproducibility, and the efficient handling of large datasets. This section explores the various methods employed for data acquisition and analysis in contemporary laboratory settings, highlighting the transition from traditional manual methods to automated systems.
Modern laboratories employ a variety of methods for collecting and storing scientific data. These range from traditional pen-and-paper notebooks to sophisticated, fully automated systems capable of collecting and processing vast amounts of data in real-time. The choice of method depends heavily on the type of experiment, the volume of data generated, and the desired level of precision and automation.
Advancements in science laboratory technology are constantly reshaping research capabilities. A key player in providing innovative solutions for various industries, including scientific research, is cherry technologies , whose contributions to automation and precision instrumentation are noteworthy. This ultimately leads to more efficient and accurate results within the scientific laboratory setting, furthering our understanding of the world around us.
Traditional Manual Data Recording versus Modern Automated Systems
The contrast between traditional manual data recording and modern automated systems is significant. While manual methods offer a certain degree of direct control and simplicity, automated systems provide advantages in terms of speed, accuracy, and data management. A comparison of the two reveals key differences.
Modern science laboratory technology relies heavily on sophisticated instruments and data analysis. The efficient management and interpretation of this data is intrinsically linked to advancements in information or technology , particularly in areas like data storage and AI-driven analysis. Ultimately, improvements in information technology directly translate to enhanced capabilities and discoveries within the scientific laboratory setting.
Traditional manual data recording, often involving handwritten notes and spreadsheets, presents several advantages and disadvantages:
- Advantages: Simple to implement; requires minimal specialized equipment; allows for immediate observation and interpretation of data.
- Disadvantages: Prone to human error; time-consuming; difficult to manage large datasets; limited analysis capabilities; data is not easily shared or backed up.
In contrast, modern automated systems offer a different set of advantages and disadvantages:
- Advantages: High accuracy and precision; increased speed and efficiency; reduced human error; facilitates data sharing and collaboration; enables advanced data analysis; better data management and security; allows for real-time monitoring and control of experiments.
- Disadvantages: Higher initial cost; requires specialized training and expertise; potential for equipment malfunction; data may be vulnerable to software errors or hacking; may lack the flexibility of manual methods for certain types of experiments.
Software for Data Analysis in Different Scientific Fields
Specialized software plays a crucial role in analyzing the data collected in scientific experiments. The choice of software depends on the specific scientific discipline and the type of data being analyzed. The following table provides examples of software commonly used in biology, chemistry, and physics.
Scientific Field | Software Examples | Description |
---|---|---|
Biology | ImageJ, Bioconductor, Geneious Prime | ImageJ is used for image analysis; Bioconductor provides tools for genomic data analysis; Geneious Prime is used for sequence alignment and analysis. |
Chemistry | ChemDraw, Mestrenova, OriginPro | ChemDraw is used for drawing chemical structures; Mestrenova is used for NMR data processing; OriginPro is used for data visualization and analysis. |
Physics | MATLAB, Igor Pro, LabVIEW | MATLAB is a powerful tool for numerical computation and data analysis; Igor Pro is used for signal processing and data visualization; LabVIEW is used for instrument control and data acquisition. |
Safety and Security in Science Laboratories
Maintaining a safe and secure science laboratory environment is paramount for protecting personnel, preserving valuable research, and ensuring compliance with regulations. The potential for accidents and security breaches necessitates a comprehensive approach encompassing robust safety protocols and stringent security measures. This section will explore key aspects of laboratory safety and security, emphasizing practical applications and preventative strategies.
Handling Hazardous Materials: Safety Protocols
Safe handling of hazardous materials is fundamental to laboratory safety. This involves a multi-layered approach, starting with proper training for all personnel. Training should cover the identification of hazards (using Safety Data Sheets or SDS), the selection and use of appropriate personal protective equipment (PPE), such as gloves, lab coats, eye protection, and respirators, and the correct procedures for handling, storage, and disposal of chemicals, biological agents, and other hazardous materials. Furthermore, well-defined spill response plans are crucial, detailing steps to take in the event of accidental spills or releases. These plans should specify the appropriate cleanup procedures for each type of hazardous material, including the use of neutralizing agents and proper waste disposal methods. Regular safety inspections and drills reinforce safe practices and identify potential hazards before incidents occur. For instance, a microbiology lab might conduct regular checks of autoclaves to ensure sterilization effectiveness and perform mock spill drills to evaluate the team’s response capabilities.
Secure Laboratory Design: Preventing Unauthorized Access
The design of a secure science laboratory plays a crucial role in preventing unauthorized access and protecting sensitive research. Physical security measures are vital, including restricted access points with keycard or biometric entry systems. Surveillance systems, such as CCTV cameras, can monitor activity within the lab and deter unauthorized entry. Secure storage for hazardous materials and valuable equipment is also necessary, using locked cabinets, safes, or specialized storage units. Additionally, robust computer security measures are essential to protect electronic data and prevent cyberattacks. This includes strong passwords, firewalls, intrusion detection systems, and regular software updates. For example, a chemistry lab might utilize a dedicated server room with access control and environmental monitoring to safeguard sensitive research data. Furthermore, controlled access to specific areas within the lab, based on personnel roles and responsibilities, can further enhance security.
Microbiology Laboratory Safety Checklist
Before commencing any work in a microbiology laboratory, a comprehensive safety checklist should be reviewed and followed meticulously. This checklist ensures adherence to safety standards and minimizes risks.
The following checklist Artikels essential safety measures:
- Proper sterilization of all equipment and work surfaces before and after use.
- Use of appropriate biological safety cabinets (BSCs) for handling infectious agents.
- Strict adherence to aseptic techniques to prevent contamination.
- Proper disposal of biohazardous waste in designated containers.
- Regular monitoring and maintenance of safety equipment, including autoclaves and incubators.
- Appropriate personal protective equipment (PPE) including gloves, lab coats, and eye protection worn at all times.
- Emergency eye wash stations and safety showers readily accessible.
- Detailed understanding and implementation of the lab’s emergency response plan.
- Regular safety training for all personnel.
- Documentation of all procedures and any safety incidents.
The Role of Robotics in Science Labs
The integration of robotic systems into scientific laboratories is rapidly transforming research and development across various disciplines. Automation of repetitive tasks, increased precision, and enhanced throughput are just some of the benefits driving this technological shift. Robotic systems are proving invaluable in streamlining workflows and enabling scientists to focus on higher-level analysis and interpretation of data.
Applications of Robotic Systems in Automating Repetitive Laboratory Tasks
Robotic systems excel at automating a wide array of repetitive tasks, significantly improving efficiency and reducing human error. These tasks often involve precise movements and meticulous procedures, areas where robots consistently outperform human capabilities. Examples include liquid handling (e.g., dispensing, mixing, diluting), sample preparation (e.g., weighing, grinding, homogenizing), and plate handling (e.g., transferring samples between plates, cell seeding). The use of robotics in these applications allows for increased throughput, reduced turnaround time for experiments, and improved reproducibility of results. Furthermore, the elimination of repetitive manual tasks frees up researchers to focus on more intellectually demanding aspects of their work.
Types of Laboratory Robots and Their Functionalities
Several types of laboratory robots are available, each designed for specific tasks and functionalities. Liquid handling robots are prevalent, using automated pipetting systems to accurately transfer liquids in various volumes. These robots are commonly used in high-throughput screening, genomics, and proteomics research. Another category is robotic arms, which offer greater flexibility and reach, enabling more complex manipulations, such as sample loading and unloading from instruments or moving objects within a laboratory environment. These are often used in conjunction with other robotic systems or integrated into larger automated workflows. Finally, specialized robots are designed for tasks like cell culturing or microscopy, optimizing specific processes with tailored designs and software. The choice of robot depends heavily on the specific needs of the laboratory and the types of experiments being conducted.
Design of a Robotic System for Cell Culturing
A robotic system for automated cell culturing could incorporate a robotic arm with a specialized end-effector for handling cell culture plates. The system would include a vision system to accurately locate and identify individual wells within the plates. Software would control the robot’s movements, allowing for precise dispensing of media, reagents, and cells into each well. A temperature-controlled incubator would be integrated into the system to maintain optimal growth conditions. The system could also incorporate sensors to monitor cell growth and viability, triggering alerts or adjustments as needed. Such a system would drastically increase the throughput of cell culture experiments, enabling higher-throughput screening of drugs or other compounds and reducing the risk of human error in the process. This automated system would also provide consistent and reproducible results across multiple experiments, improving the reliability of research findings. The design could further incorporate features like automated waste disposal and cleaning cycles to maintain a sterile environment.
Laboratory Information Management Systems (LIMS)
Laboratory Information Management Systems (LIMS) are software solutions designed to manage and track laboratory data and workflows. They streamline operations, improve data integrity, and enhance overall laboratory efficiency across diverse scientific disciplines. From sample management to reporting, LIMS software plays a critical role in modern laboratory operations.
LIMS software functionality encompasses a wide range of features aimed at optimizing laboratory processes. Core functionalities typically include sample management (tracking samples from receipt to disposal), instrument integration (connecting to analytical instruments for automated data transfer), quality control (monitoring and managing quality assurance processes), data analysis (providing tools for data interpretation and reporting), and reporting (generating customized reports for various stakeholders). Advanced LIMS platforms may also include features such as inventory management, regulatory compliance tools, and electronic signature capabilities.
LIMS Platform Comparison, Science laboratory technology
Different LIMS platforms offer varying features and capabilities tailored to specific laboratory needs and budgets. Some platforms are designed for small, specialized labs with limited sample volumes, while others cater to large, complex facilities handling high sample throughput and diverse analytical techniques. Key factors to consider when comparing LIMS platforms include scalability (ability to handle increasing sample volumes and data), integration capabilities (compatibility with existing laboratory instruments and systems), user interface (ease of use and intuitiveness), regulatory compliance (adherence to relevant industry standards), and cost (licensing fees, implementation costs, and ongoing maintenance). Open-source LIMS solutions also exist, offering flexibility and customization but often requiring more technical expertise for implementation and maintenance.
Examples of LIMS Efficiency Improvements
LIMS implementation significantly enhances efficiency and data management in various laboratory settings. For instance, in clinical diagnostic labs, LIMS streamlines patient sample tracking, reduces manual data entry errors, and accelerates turnaround times for test results. This translates to improved patient care and faster diagnosis. In environmental testing labs, LIMS improves the management of environmental samples, ensuring accurate tracking of sample location, chain of custody, and analytical results. This leads to better environmental monitoring and more reliable data for regulatory reporting. Similarly, in pharmaceutical research labs, LIMS supports efficient management of drug discovery and development processes, improving data traceability and compliance with regulatory requirements. This accelerates the drug development pipeline and reduces the risk of errors. The implementation of LIMS in food safety labs ensures traceability throughout the food production chain, enhancing the safety and quality of food products and improving response times to potential contamination incidents.
Impact of Technology on Scientific Research
Technological advancements have fundamentally reshaped the landscape of scientific research, accelerating the pace of discovery and pushing the boundaries of human knowledge in unprecedented ways. The integration of sophisticated instruments, computational tools, and data analysis techniques has not only enhanced the efficiency of research but also opened up entirely new avenues of investigation previously inaccessible to scientists. This transformative impact is evident across diverse scientific disciplines, from genomics and materials science to astrophysics and climate research.
The synergy between technological innovation and scientific progress is a powerful engine driving groundbreaking discoveries. Advanced technologies provide researchers with the tools to observe, measure, and analyze phenomena with unparalleled precision and scale, leading to a more comprehensive and nuanced understanding of the natural world. This enhanced capability translates into faster research cycles, increased data output, and ultimately, more rapid scientific breakthroughs.
Groundbreaking Discoveries Enabled by Advanced Laboratory Technologies
The Human Genome Project, completed in 2003, stands as a prime example of a scientific endeavor profoundly impacted by technological advancements. The project’s success hinged on the development of high-throughput DNA sequencing technologies, which enabled researchers to map the entire human genome far more quickly and efficiently than would have been possible using previous methods. This achievement has revolutionized our understanding of human genetics, paving the way for personalized medicine and advancements in disease diagnosis and treatment. Similarly, the development of advanced microscopy techniques, such as cryo-electron microscopy (cryo-EM), has allowed scientists to visualize biological molecules with atomic-level resolution, leading to breakthroughs in structural biology and drug discovery. Cryo-EM, for instance, has enabled the detailed visualization of complex protein structures, providing crucial insights into their function and interactions. This has been instrumental in the development of new therapies targeting specific proteins involved in diseases like Alzheimer’s and cancer.
Technology Investment and Research Output in Genomics
The relationship between technology investment and research output is particularly striking in the field of genomics. Increased funding for the development of next-generation sequencing (NGS) technologies has led to a dramatic increase in the volume of genomic data generated, enabling large-scale genomic studies that were previously infeasible. For example, the cost of sequencing a human genome has plummeted from billions of dollars in the early 2000s to less than a thousand dollars today, primarily due to technological advancements in NGS platforms. This reduction in cost has facilitated widespread adoption of genomic technologies in various research areas, leading to a surge in publications and discoveries related to human genetics, disease mechanisms, and evolutionary biology. The exponential growth in genomic data has also fueled the development of powerful bioinformatics tools and algorithms, further accelerating the pace of discovery within this field. This positive feedback loop demonstrates the powerful synergy between technological investment and scientific progress.
Virtual and Remote Laboratories
Virtual and remote laboratories (VRLs) are transforming science education and research by providing accessible, cost-effective, and often safer alternatives to traditional physical labs. They offer significant advantages, particularly in situations where access to physical equipment is limited or where the experiments themselves pose safety risks. However, challenges remain in replicating the full tactile and experiential learning of a physical lab.
VRLs utilize software simulations and remote access to physical equipment to allow users to conduct experiments and collect data from anywhere with an internet connection. This approach has implications for both education and research, impacting how scientific concepts are taught and how research is conducted.
Benefits and Challenges of Virtual and Remote Laboratory Environments
The benefits of VRLs are numerous. They offer increased accessibility to students and researchers regardless of geographical location or resource limitations. The cost-effectiveness is also significant, as VRLs reduce the need for expensive equipment and consumables. Furthermore, VRLs can enhance safety by allowing students to perform potentially hazardous experiments in a controlled virtual environment. However, challenges include the potential for a lack of hands-on experience, the need for reliable internet connectivity, and the potential for software limitations to restrict the scope of experiments that can be performed. The digital divide also presents a barrier to equitable access, particularly for students and researchers in under-resourced areas.
Comparison of Physical and Virtual Laboratories
Physical laboratories provide an unparalleled hands-on experience, allowing for direct interaction with equipment and materials. This direct interaction fosters a deeper understanding of experimental procedures and allows for unexpected observations and discoveries. However, physical labs are expensive to establish and maintain, require significant space, and may present safety hazards. In contrast, virtual labs are more cost-effective and safer, but they may lack the tactile experience and flexibility of a physical lab. For instance, a chemistry experiment involving titration would allow for precise control and visual observation in a physical lab, while a virtual simulation might offer simplified representations of the process, potentially lacking the nuances of real-world observations. Similarly, a physics experiment involving the study of simple harmonic motion might benefit from the immediate feedback of a physical pendulum in a physical lab compared to the simulated response of a virtual pendulum.
Software and Hardware Requirements for a Functional Remote Laboratory Setup
Establishing a functional remote laboratory requires a combination of specialized software and hardware. On the software side, a robust virtual laboratory platform is essential. This platform typically includes software simulations of experiments, data acquisition tools, and collaborative features for communication and data sharing. Examples of such platforms include Labster, PhET Interactive Simulations, and various custom-built solutions developed by universities and research institutions. Hardware requirements depend on the complexity of the experiments. At a minimum, a computer with sufficient processing power and memory is necessary, along with a reliable internet connection. For remote access to physical equipment, specialized hardware such as cameras, sensors, and actuators may be required, along with a control system to manage the equipment remotely. In some cases, dedicated servers and network infrastructure are needed to support multiple users and ensure data security. For example, a remote robotics lab might require specialized robotic arms, cameras for visual feedback, and powerful computers to process the data and control the robot’s actions. Similarly, a remote chemistry lab might require specialized sensors for measuring pH, temperature, and conductivity, all integrated into a system that can be controlled and monitored remotely.
Laboratory Sustainability and Green Technology
Modern science laboratories, while crucial for advancements, often have a significant environmental footprint. The pursuit of sustainability in these spaces is no longer optional but a necessity, demanding a shift towards environmentally friendly practices and technologies. This involves minimizing waste generation, reducing energy and water consumption, and promoting the use of safer chemicals.
Implementing green technologies in laboratories requires a multifaceted approach, encompassing both operational changes and the adoption of innovative equipment. This section will explore some key aspects of creating a more sustainable laboratory environment.
Energy-Efficient Equipment and Practices
Many laboratory instruments are significant energy consumers. Switching to energy-star certified equipment, such as refrigerators, centrifuges, and incubators, is a crucial first step. Implementing energy management systems that monitor and control energy usage in real-time can further optimize consumption. Simple practices, such as turning off equipment when not in use and utilizing timers, also contribute significantly to energy savings. For example, a laboratory that replaces its older, less efficient autoclave with a newer model can see a substantial reduction in its electricity bill and carbon footprint. Furthermore, optimizing the cooling system through regular maintenance and the use of efficient HVAC systems plays a vital role.
Waste Reduction and Management Strategies
Waste generation is a major concern in scientific laboratories. Implementing a robust waste management plan, including proper segregation and recycling programs for different types of waste (chemical, biological, glass, plastic, etc.), is essential. Adopting techniques that minimize the use of hazardous chemicals, such as using safer substitutes or employing microfluidic devices that require smaller sample volumes, also significantly reduces waste. A robust inventory management system can prevent unnecessary purchases and reduce the accumulation of expired chemicals. For instance, a laboratory that switches to a closed-loop system for solvent recycling can dramatically reduce its hazardous waste disposal costs and environmental impact.
Water Conservation Measures
Laboratories often consume considerable amounts of water. Implementing water-saving fixtures, such as low-flow faucets and sinks, can significantly reduce water consumption. Designing experiments to minimize water usage and reusing water where possible (e.g., in rinsing steps) are additional strategies. Consider installing water purification systems that reuse purified water instead of constantly drawing fresh water. A specific example is the implementation of a reverse osmosis system to recycle and purify wastewater, minimizing water waste and reducing the overall water footprint of the laboratory.
Sustainable Chemical Selection and Use
Choosing environmentally friendly chemicals is paramount. Prioritizing less toxic and biodegradable alternatives whenever possible significantly reduces the environmental burden. Adopting greener synthesis methods, such as microwave-assisted or ultrasound-assisted reactions, often reduces the amount of solvent used and improves reaction efficiency. Furthermore, implementing robust safety protocols and training programs for personnel ensures the safe handling and disposal of chemicals. For example, a switch from traditional solvents to supercritical CO2 in extraction processes reduces both the use of volatile organic compounds and the volume of waste generated.
The Future of Science Laboratory Technology
The next decade promises a transformative shift in science laboratory technology, driven by converging advancements in artificial intelligence, automation, miniaturization, and data analytics. These changes will not only enhance research efficiency and accuracy but also reshape the accessibility and societal impact of scientific discovery. We can anticipate a future where laboratories are more intelligent, interconnected, and environmentally conscious.
Automation and Artificial Intelligence in Laboratories
Automation is already prevalent in many labs, but the future will see a dramatic increase in its sophistication and integration. AI-powered robots will perform complex tasks like sample preparation, analysis, and data interpretation with greater speed and precision than human operators. For example, automated liquid handling systems combined with AI-driven image analysis could revolutionize drug discovery by enabling high-throughput screening of thousands of compounds daily. This level of automation will free up scientists to focus on higher-level tasks like experimental design and data interpretation, accelerating the pace of research. Furthermore, AI algorithms can identify patterns and anomalies in data that might be missed by human researchers, leading to novel discoveries.
Miniaturization and Microfluidics
Miniaturization will continue to be a key driver of innovation. Microfluidic devices, which manipulate small volumes of fluids, offer significant advantages in terms of cost, efficiency, and waste reduction. Imagine lab-on-a-chip devices capable of performing complex diagnostic tests using only a drop of blood, enabling rapid and point-of-care diagnostics in remote areas. This technology will have a profound impact on healthcare, environmental monitoring, and personalized medicine. The reduction in reagent consumption also contributes to a greener and more sustainable laboratory environment.
Advanced Data Acquisition and Analysis
The volume of data generated in scientific research is exploding. Future laboratories will rely on sophisticated data management and analysis tools to handle this influx. Cloud-based platforms will enable seamless data sharing and collaboration among researchers worldwide. Advanced analytical techniques, such as machine learning and deep learning, will be used to extract meaningful insights from complex datasets, accelerating the pace of discovery and potentially leading to breakthroughs in fields like genomics and materials science. For example, AI-powered image analysis software can already identify specific cells or molecules within microscopic images, significantly speeding up the process of data interpretation in fields like pathology and biology.
A Vision for the State-of-the-Art Science Laboratory
A state-of-the-art science laboratory of the future will be a highly integrated and intelligent environment. It will feature automated systems for sample handling, analysis, and data management, seamlessly integrated with AI-powered tools for data interpretation and experimental design. The lab will be highly sustainable, utilizing energy-efficient equipment and minimizing waste generation. Safety will be paramount, with integrated monitoring systems and automated safety protocols. Remote access and collaboration will be commonplace, allowing scientists to conduct experiments and analyze data from anywhere in the world. For example, a researcher in a remote location could remotely control a robotic arm in a specialized laboratory to perform a complex experiment, analyze the results, and share the data with collaborators globally. This level of accessibility will democratize scientific research and empower scientists in under-resourced areas. Furthermore, the integration of virtual and augmented reality technologies will enhance training and education, providing immersive and interactive learning experiences for students and researchers.
Closing Summary
In conclusion, the ongoing advancements in science laboratory technology are not merely enhancing efficiency; they are fundamentally reshaping the landscape of scientific research. The integration of automation, artificial intelligence, and sustainable practices is creating a new era of discovery, enabling scientists to tackle complex challenges with unprecedented precision and speed. As technology continues to evolve, the future of scientific exploration promises even greater breakthroughs, driven by the innovative tools and techniques explored within this discussion.